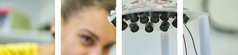
Pretty tiny – from individual electrons to nanoparticles
From individual electrons to new electrochemical applications
Professor Axel Lorke and Dr Paul Geller were able to trace the movement of individual electrons through an electronic component within the scope of several DFG-funded projects. Minuscule semiconductor nanoparticles called ‘quantum dots’ display characteristic optical properties depending on their charge state. A single electron determines whether a quantum dot emits light when exposed to laser irradiation. If its charge state changes, the dot produces a characteristic flashing pattern, which shows whether it has just captured or emitted an electron. Professor Jürgen König’s research group has evaluated these ‘optical telegraph signals’ together with the CRC 1242 team.
Metal nanoparticles made from platinum are used to convert chemical into electrical energy, for example, in fuel cells or when generating hydrogen as a power source. For the EU-funded project ‘MoreInnoMat’, Dr Nicolas Wöhrl of Professor Axel Lorke’s research group successfully worked with Professor Stephan Schulz’s (Chemistry) group on developing a process for synthesising carbon nanowalls with embedded platinum nanoparticles. In the process, for which a patent application has been filed, two-dimensional carbon atom layers (graphene) are closely connected to platinum nanoparticles, which makes the structure particularly sturdy for practical application.
Nanoscale magnetic systems
Professor Michael Farle’s research group studies the properties of nanoscale magnetic systems. Synthesis of new materials has enabled a variety of new applications, such as the use of efficient permanent magnets in electric motors and magnetocaloric materials in innovative cooling technologies. The researchers are studying these and other applications intensively within the scope of the new DFG Transregio CRC/TRR 270, working together with colleagues from TU Darmstadt. Besides nanoparticles for additive manufacturing (3D printing), they are producing special magnetic Heusler compounds, in which small ferromagnetic particles (< 2 nm) in an antiferromagnetic matrix exhibit very high coercivity at room temperature.
Researchers in the DFG project ‘Magnetic Landscapes’ use laterally periodically modulated magnetisation to examine ultra-thin layers and control spin wave phenomena in the sub-terahertz range. Another key research area is the development of hybrid nanoparticles for medical theranostics. This is the focus of the EU-funded ‘MaNaCa’ project, a collaborative endeavour involving colleagues from Greece and Armenia. Its combination of therapy and diagnostics in magnetic nanoparticles paves the way for new approaches to treating cancer.
The effect of the hot electron
You may not really see them, but you can still trace the energy flow with your naked eye. It’s a bit like thumbing through a flip book: a team of scientists from the groups of Professor Uwe Bovensiepen, Professor Rossitza Pentcheva and Professor Heiko Wende has investigated energy transfer in a metal insulator material. In the long term, their findings may contribute to a solution for the problem of heat generation in microelectronics through precise material design. Any attempt to get to the bottom of this phenomenon will inevitably lead to the atomic level and, as such, to electrons making their way through various materials. But how exactly do they do this?
That is what UDE physicists are studying in CRC 1242 ‘Non-Equilibrium Dynamics of Condensed Matter in the Time Domain’. They have analysed the layer structures of the metal insulator material using a pump-probe process. A laser pulse feeds energy into the system. This energy stimulates the electrons and ‘heats them up’, so to speak. Soon after, an X-ray determines from a snapshot how the ‘hot’ electrons spread through the material.
The result: the hot electrons stimulate the metal lattice within less than a picosecond. Almost simultaneously, the interface between the materials begins to oscillate. Surprisingly, the insulator reacts just a picosecond later. Theoretical simulations have confirmed the significance of interface oscillations.
Nanoparticles in the quantum regime
Quantum physics describes the microcosm perfectly. If we apply it to large objects rather than to atoms or photons, however, it tend to produce predictions that turn our traditional views of the world upside down. According to these predictions, a single object should be able to exist simultaneously in multiple places and even change its behaviour depending on whether or not it is observed. Professor Klaus Hornberger’s research group studies systems that straddle the boundary between this quantum regime and traditional physics.
One of its research highlights in the past two years was the quantum-mechanical description of nanoparticles that are suspended by laser light and, as such, largely uninfluenced by environmental factors. The researchers have recently developed a feasible method of putting such a nanoparticle into the quantum-mechanical basic state of its centre-of-mass motion and rotational motion, i.e., cooling it to absolute zero. This method may be a point of departure for future fundamental experiments and technological applications on which the research group is working.
Electron tunnelling in quantum dots
The flow of electrical currents through conventional circuits involves a vast number of electrons. When circuits are realised in nanostructures, i.e., made very small, however, the current can be transported by much fewer electrons. During the transport of current through a quantum dot, individual electrons exhibit the tunnel effect: they successively jump back and forth between the electricity supply and the quantum dot. Such quantum jumps constitute the smallest possible unit of current transport. Their measurement provides the greatest possible amount of information that can be extracted from the system.
Professor Jürgen König’s group develops theoretical tools that makes it possible to analyse electron tunnelling in quantum dots statistically in order to obtain important information about the underlying system. A highlight of this project has been the application of their theory to experiments carried out in Professor Axel Lorke’s research group, in which electron tunnelling in quantum dots was measured with high precision using time-resolved methods. This allowed the researchers to determine the spin-relaxation time of an electron in a quantum dot among other insights.